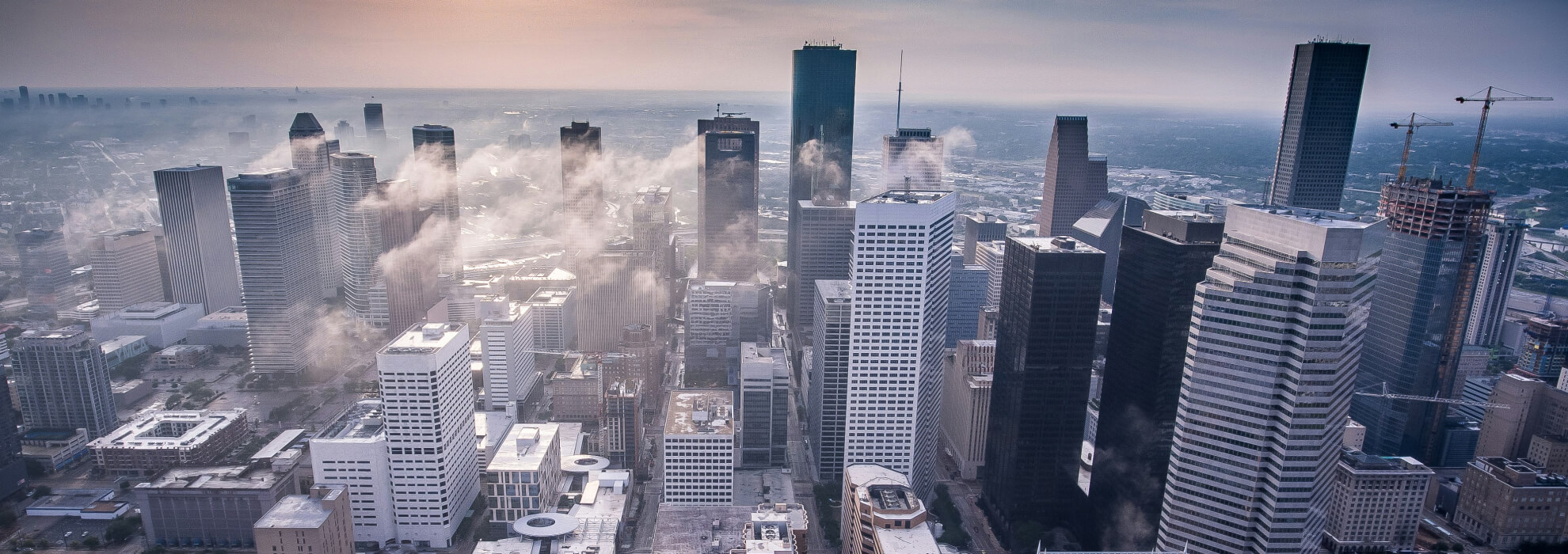
Watch short for this article (5 slides)
Beyond Emission Cuts: Direct Air Capture and the Quest to Utilize Atmospheric CO₂
As the urgency of the climate crisis intensifies, strategies are expanding beyond solely reducing greenhouse gas emissions. While slashing emissions remains paramount, technologies designed to actively remove existing carbon dioxide (COâ‚‚) from the atmosphere are gaining prominence. Among the most discussed is **Direct Air Capture (DAC)** – an innovative approach that chemically scrubs COâ‚‚ directly from the ambient air. Often linked with long-term carbon storage, DAC also unlocks intriguing possibilities for **Carbon Capture and Utilization (CCU)**, transforming captured COâ‚‚ from a liability into a potential feedstock for valuable products across diverse industries. This article explores the mechanics of DAC technology, the burgeoning field of carbon utilization, the critical challenges involved, and the potential role of DAC/CCU in a sustainable future.
What is Direct Air Capture (DAC) and Why Do We Need It?
Direct Air Capture refers to technologies designed to capture COâ‚‚ directly from the atmosphere, where its concentration is very low (currently around 420 parts per million, or 0.042%), unlike capturing it from concentrated point sources like power plants or industrial facilities (where COâ‚‚ can be 10-15% or higher).
The need for DAC arises from several key climate realities:
- Legacy Emissions: Billions of tons of COâ‚‚ emitted over decades remain in the atmosphere, contributing to ongoing warming. Reducing current emissions to zero doesn't remove this historical burden.
- Hard-to-Abate Sectors: Some industries (e.g., cement production, long-haul aviation, heavy industry) face significant challenges in completely eliminating their emissions in the near term. DAC offers a potential pathway to offset these residual emissions.
- Net-Zero Goals & Net-Negative Emissions: Achieving global net-zero emissions targets likely requires not only drastic emission cuts but also active carbon dioxide removal (CDR) to balance out any remaining emissions. To eventually lower atmospheric COâ‚‚ concentrations, net-negative emissions (removing more COâ‚‚ than is emitted) will be necessary, making technologies like DAC potentially crucial. (IPCC AR6 Working Group III Report - Mitigation of Climate Change)
How DAC Technology Works: Pulling COâ‚‚ From Thin Air
Capturing COâ‚‚ from ambient air is thermodynamically challenging due to its dilute nature. Current DAC technologies primarily fall into two main categories:
- Solid DAC (S-DAC):
- Mechanism: These systems use solid sorbent materials that chemically bind with COâ‚‚. Large fans push ambient air through filters or structures coated with these sorbents. Once the sorbent is saturated with COâ‚‚, it needs to be regenerated to release the captured COâ‚‚ for collection.
- Regeneration: This typically involves heating the sorbent (Temperature Swing Adsorption - TSA) or changing the pressure (Pressure Swing Adsorption - PSA or Vacuum Swing Adsorption - VSA) to break the chemical bond and release concentrated COâ‚‚ gas.
- Example Players/Concepts: Companies like Climeworks utilize solid amine-functionalized sorbents regenerated with low-grade heat (around 80-100°C).
- Liquid DAC (L-DAC):
- Mechanism: These systems pass air through chemical solutions (e.g., aqueous potassium hydroxide or sodium hydroxide) that absorb COâ‚‚. The COâ‚‚ reacts with the solution to form carbonate salts.
- Regeneration: The carbonate solution is processed through a chemical loop, often involving heating in a calciner (similar to those used in cement or pulp production) at high temperatures (around 900°C), to release the COâ‚‚ in a pure stream and regenerate the original chemical solvent for reuse.
- Example Players/Concepts: Companies like Carbon Engineering (now part of Occidental Petroleum) employ this liquid solvent approach.
Both approaches require significant energy input, primarily for moving large volumes of air and, crucially, for regenerating the sorbents/solvents to release the captured COâ‚‚.
Beyond Storage: Innovative Applications for Captured COâ‚‚ (Carbon Utilization)
Source: Nature.org
While permanently storing captured COâ‚‚ deep underground (geological sequestration) is a primary option for climate mitigation (often termed DACCS - Direct Air Capture and Carbon Storage), the captured COâ‚‚ can also be used as a feedstock – Carbon Capture and Utilization (CCU).
"Turning atmospheric COâ‚‚ from a waste product into a valuable input for new products could create new markets and potentially offset some of the high costs associated with direct air capture." - Perspective based on IEA reports on CCUS.
Key utilization pathways include:
- Sustainable Aviation Fuels (SAFs) & E-Fuels (Power-to-X): Captured COâ‚‚ can be combined with "green" hydrogen (produced via electrolysis using renewable electricity) through processes like Fischer-Tropsch synthesis or methanol synthesis to create synthetic liquid fuels (e-fuels) or gases. These fuels can be chemically similar to conventional jet fuel, diesel, or gasoline but have a potentially much lower lifecycle carbon footprint if produced using clean energy. This is particularly promising for decarbonizing hard-to-abate sectors like long-haul aviation and shipping. (IEA - Aviation Sector Overview)
- Building Materials (COâ‚‚ Mineralization): Captured COâ‚‚ can be reacted with minerals (like calcium and magnesium oxides, often found in industrial wastes like steel slag or quarried rock) or injected into concrete during curing. This process, called mineralization, converts gaseous COâ‚‚ into stable solid carbonates (e.g., calcium carbonate - limestone), effectively locking the carbon away permanently within building materials like concrete blocks or aggregates. This can potentially displace emissions-intensive conventional cement production and create carbon-negative concrete. Companies like CarbonCure and Solidia Technologies are active in this space.
- Chemicals and Polymers: COâ‚‚ can serve as a carbon feedstock for producing various chemicals, including methanol (a platform chemical), formic acid, urea (for fertilizers), and polymers (plastics). This offers a pathway to displace fossil fuel feedstocks in the chemical industry.
- Enhanced Plant Growth: Injecting concentrated COâ‚‚ into greenhouses can significantly boost photosynthesis and increase crop yields for certain plants (e.g., tomatoes, cucumbers, flowers). While a valid use, the scale is limited compared to the amount of COâ‚‚ needing removal for climate purposes.
- Food and Beverage Industry: Carbonation of beverages is a long-standing use of COâ‚‚, though the volume required is relatively small compared to potential fuel or material applications. COâ‚‚ is also used in food processing and packaging.
- Other Niche Applications: Including enhanced oil recovery (EOR - though its climate benefit is debated as it facilitates fossil fuel extraction), fire suppression systems, dry ice production, and certain medical applications.
Utilization vs. Storage: The Critical Question of Permanence
When evaluating the climate benefit of DAC and CCU, the **permanence** of the carbon removal is paramount:
- Storage (DACCS): Geological storage aims to lock COâ‚‚ away for millennia, offering true carbon dioxide removal from the atmosphere-ocean system.
- Utilization (CCU): The climate benefit depends heavily on the product's lifespan and end-of-life fate.
- Long-Lived Products (e.g., Mineralized Concrete): Effectively permanent storage, providing clear climate benefits.
- Short-Lived Products (e.g., E-fuels, Beverages): Using COâ‚‚ in fuels means it is released back into the atmosphere upon combustion. While this can create a *carbon-neutral cycle* (displacing fossil fuel emissions), it does *not* represent net carbon dioxide removal unless the fuel production itself is highly efficient and powered entirely by clean energy. Using COâ‚‚ for carbonation quickly returns it to the atmosphere.
Therefore, rigorous **lifecycle assessments (LCAs)** are crucial to determine the true climate benefit of CCU pathways, accounting for the energy used in capture, conversion, and the product's lifespan.
Overcoming the Hurdles: Challenges Facing DAC and CCU
Despite the potential, significant obstacles remain:
- High Costs: DAC is currently very expensive. Estimates vary widely depending on the technology, scale, and energy source, but costs are often cited in the range of **$200 to $1000+ per ton of COâ‚‚ captured**. This is significantly higher than capturing COâ‚‚ from point sources. Substantial cost reductions through innovation and scale-up are needed for widespread deployment.
- Massive Energy Requirements: Both DAC capture and subsequent utilization processes (especially e-fuel synthesis) are energy-intensive. To be climate-beneficial, this energy **must** come from low-carbon or zero-carbon sources (renewables, nuclear, potentially fossil fuels with very high carbon capture rates). Using fossil fuel-generated energy for DAC could result in more COâ‚‚ being emitted than captured.
- Scalability Challenges: Achieving climate relevance requires DAC deployment on a massive scale – removing millions, eventually billions, of tons of COâ‚‚ per year. This requires significant land use (for facilities and renewable energy generation), water resources, supply chains for materials (sorbents, chemicals), and infrastructure for COâ‚‚ transport and storage/utilization.
- Policy and Market Development: Strong policy support is needed, including carbon pricing, subsidies/tax credits (like the 45Q tax credit in the US), public procurement mandates, reliable carbon accounting frameworks, and the development of stable markets for COâ‚‚-derived products.
- Monitoring, Reporting, and Verification (MRV): Robust MRV systems are essential to accurately quantify the amount of COâ‚‚ captured, stored, or utilized and ensure the permanence and climate integrity of different pathways.
- Public Perception and Acceptance: Building public trust and addressing concerns regarding costs, land use, energy consumption, and the safety/permanence of storage/utilization are important for social license to operate.
The Road Ahead: Integrating DAC and CCU into Climate Solutions
DAC and CCU are not silver bullets but are increasingly seen as potentially necessary components of a comprehensive climate strategy, alongside aggressive emissions reductions.
- Complementary Role: Their primary role will likely be addressing legacy emissions and offsetting residual emissions from hard-to-abate sectors.
- Innovation and Investment: Continued research, development, and demonstration are crucial to improve efficiency, reduce costs, and scale up technologies responsibly.
- Synergies: Exploring synergies, such as co-locating DAC facilities with renewable energy hubs, green hydrogen production sites, or geological storage formations, can improve economics and efficiency.
- Ethical Considerations: Ensuring equitable deployment, considering land use implications, and maintaining a primary focus on emissions reduction are vital.
Conclusion: From Atmospheric Liability to Potential Asset
Direct Air Capture technology offers the groundbreaking possibility of removing historical COâ‚‚ emissions directly from the atmosphere. While permanent geological storage is key for long-term climate mitigation, the potential to utilize captured COâ‚‚ as a feedstock for fuels, building materials, chemicals, and other products presents exciting economic and industrial opportunities. However, realizing this potential requires overcoming significant hurdles in cost, energy demand, and scale, while ensuring the processes are powered by clean energy and the climate benefits are rigorously verified. As innovation accelerates and policy support grows, DAC and CCU could evolve from nascent technologies into crucial tools in the portfolio of solutions needed to achieve a stable climate and a more circular economy, transforming atmospheric carbon from solely a problem into a potential resource.